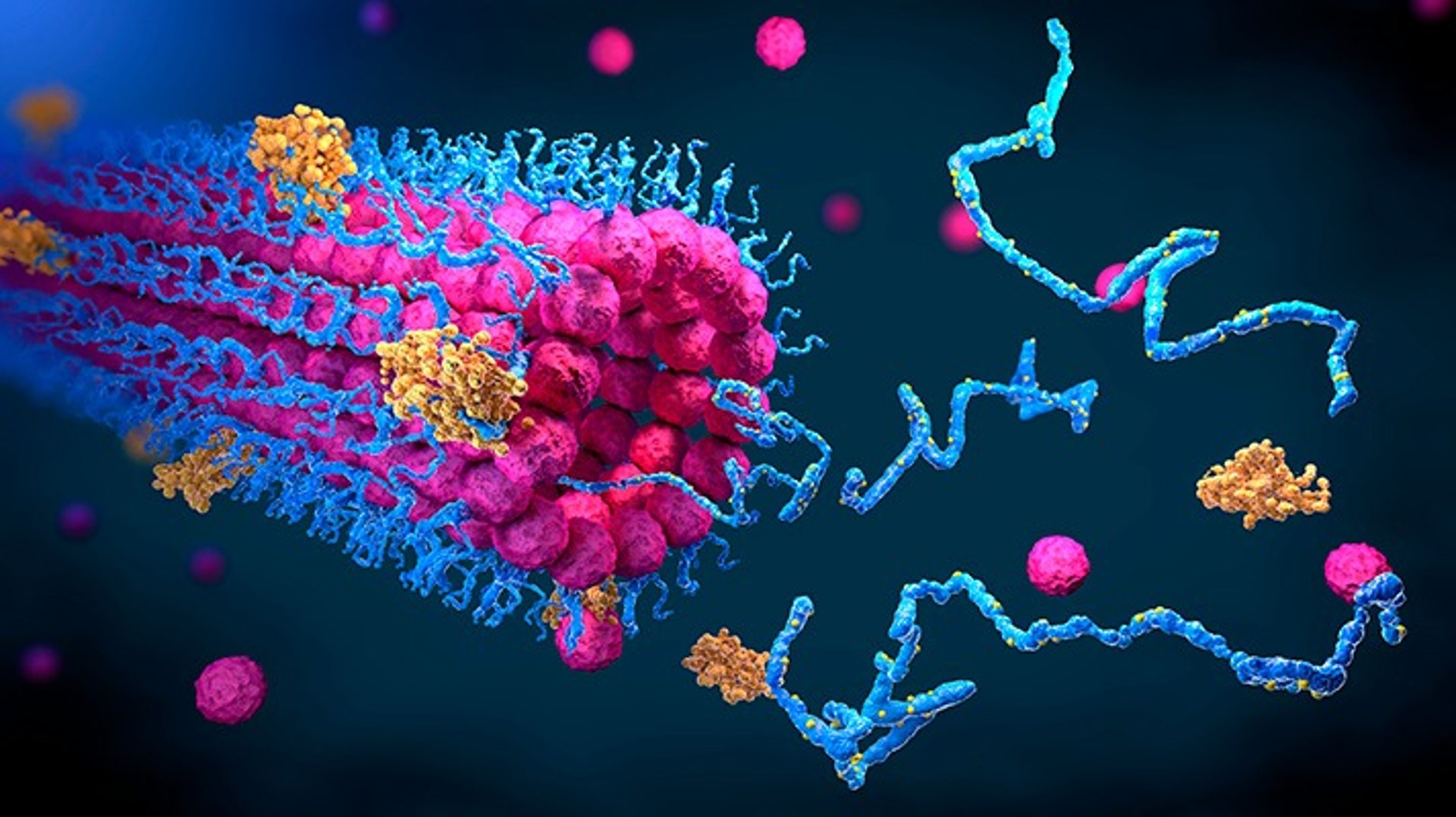
The Origami of Life
BIOLOGY
Akankshya Sahu
0rigami is a traditional Japanese art of paper folding to make models of inanimate objects, animals and flowers. Anyone can easily learn it by following illustrative instructions. But imagine that you’re told to make an origami, say of a paper crane, that too without any instruction manual. As you craft your origami, you take care that the fold and shape are proper. Well, the mechanism of protein folding is driven via similar modus operandi. A linear sequence of amino acids performs the job with so much precision that it ends up to form a stable and functional 3D structure out of innumerable conformations in a fraction of seconds.
Proteins are one of the most abundant organic molecules in living organisms. They are made up of the same fundamental building blocks – the Amino Acids, yet they come in diverse shapes, sizes & molecular weights. They are vital “molecular players” of our body, and they have their unique functions. They not only form structural parts of cellular entities but also act as transporters, cellular signals and metabolic enzymes. The primary structure of a protein is like pearls embedded on a long string which are nothing but amino acids connected by peptide bonds [1]. It folds spontaneously and is held together by several molecular interactions, which are determined by the sequence of amino acids and facilitated by the chaperones to ensure that proteins fold correctly and become thermodynamically stable to form complex levels of protein structures [2] [3]. The classic experiment by Anfinsen and his colleagues in 1950s on the protein ribonuclease is remarkable! They treated native ribonuclease with denaturating agents which break the hydrogen bonds and disulfide bridges that resulted in an unfolded structure. As a result, the protein was unable to function anymore. When the agents were removed, the denatured ribonuclease refolded spontaneously with all covalent & non covalent bonds accurately reformed and its catalytic activity restored as it was before. Thus, he postulated “Anfinsen’s Dogma” which says that the ‘protein’s amino acid sequence contains the information required to form a native structure in a standard physiological environment and it is essential for protein function.’
After all the discussion, I want you to ponder upon the question – How does a protein arrive at its native conformation? We shall have a look at a thought experiment called the “Levinthal’s Paradox” [4] [5]. Based on the magnitude of φ, ψ & ω angles around the covalent bond between subsequent amino acids (see diagram), a peptide linkage might exist in three conformations(other non- covalent interactions also contribute to differential conformations of a protein). Hence, for a polypeptide chain with 101 amino acids, i.e., 100 bonds, 3100 conformation can be theoretically configured. However, only one of these configurations corresponds to the native state leading to the functionality of the protein. Even if the protein is able to sample new configurations at a rate of 1013/second, it will take 1.6*1027 years to try them all which is beyond the probable age of the Universe [6].
It can be speculated from the above mentioned paradox that protein folding must follow a kinetically and thermodynamically controlled definitive pathway to form the native structure. The generally accepted idea is that to consider an energy landscape similar to the shape of a folding funnel with different conformations of a protein represented with their respective energies and the native conformation having the least energy is at the apex of the funnel. When a protein is folding, it will pass through formation of one conformation into another in an order of decreasing energies, going downhill the funnel, to finally form a native conformation [7] [8]. This hypothesis is ambiguous as scientists still need to experimentally determine the pathway of protein folding.
Just like we may go wrong while folding an origami paper, some mishaps may happen during protein folding leading to the loss of functionality of the misfolded protein. This happens due to genetic mutations and stressful cellular conditions[9]. Such misfolded proteins are a hallmark for several diseases like Type II Diabetes, Alzheimer’s disease, Huntington’s disease and Parkinson’s disease [10]. In these diseases, formation of harmful amyloid fibres takes place which leads to cellular dysfunction and finally, cell death.
Nowadays, the approach of getting computers on work to predict and analyze protein structures is a trend. Supercomputers like IBM Blue Gene are specially designed to simulate protein folding. Surprisingly, while sitting right now on your PC, with some simple bioinformatics tools like Rosetta@home and available databases of amino acid sequences, you can design proteins on your own! Such advancements are useful for selection of suitable proteins for research purpose, studying effects of misfolding and designing structure-based life-saving drugs for targetting diseases.
By solving the “protein folding problem” with fundamental principles and novel approaches, one day we will certainly understand the perfection of the origami that drives life.
REFERENCES
1. Sadava, D. E., Hillis, D.M., Heller, C. H., & Hacker, S.D. (2016). Life: The Science of Biology (11th Edition) W. H. Freeman.
2. Freeman, S., Quillin, K., Allison, L., Black, M., Taylor, E., Podgorski, G., & Carmichael, J. (2016). Biological Science (6thEdition) Pearson.
3. Lehninger, A. L., Nelson,4D. L., & Cox, M. M. (2017). 4.4/ Protein denaturation and folding. In Lehninger principles of biochemistry (7th ed., pp. 416-441). New York: W. H. Freeman and Company.
4. Levinthal C. How to fold graciously. Mossbauer Spectroscopy in Biological Systems: Proceedings of a meeting held at Allerton House, Monticello, Illinois. Editors: J. T. P. DeBrunner and E. MunckUniversity of Illinois Press (1969). Pages 22-24.
5. Berg, J. M., Tymoczko, J. L., Gatto, G. J., &Stryer, L. (2019). Biochemistry. New York: W.H. Freeman/McMillan Learning.
6. Kubelka, J., Hofrichter, J., & Eaton, W. A. (2004). The protein folding ‘speed limit.’ Current Opinion in Structural Biology, 14(1), 76– 88. https://doi. org/10.1016/j.sbi.2004.01.013
7. Dill, K. A., Ozkan, S. B., Shell, M. S., & Weikl, T. R. (2008). The Protein Folding Problem. Annual Review of Biophysics, 37(1), 289–316. https://doi.org/10.1146/annurev. biophys.37.092707.153558
8. Chikenji, G., Fujitsuka, Y., & Takada, S. (2006). Shaping up the protein folding funnel by local interaction: Lesson from a structure prediction study. Proceedings of the National Academy of Sciences, 103(9), 3141-3146. https://doi. org/10.1073/pnas.0508195103
9. Scior, A., Juenemann, K., & Kirstein, J. (2016). Cellular strategies to cope with protein aggregation. Essays in Biochemistry, 60(2), 153– 161. https://doi.org/10.1042/ebc20160002
10. Hayat, M. (2015). Introduction to Autophagy. Autophagy: Cancer, Other Pathologies, Inflammation, Immunity, Infection, and Aging, 1–53. https://doi.org/10.1016/ b978-0-12-801043-3.00001-7